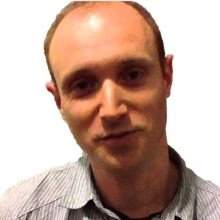
Dr Vincent Boyer
Principal investigatorDeputy director – CDT for Topological Design
+44 (0)121
414 4573
v.boyer (at) bham.ac.uk
university page
We are an experimental group in the School of Physics and Astronomy of the University of Birmingham. We use lasers interacting with atomic gases to control the behaviour of the light and the atoms at the quantum level. This enables us to engineer remarkable conditions, in which a gas of atoms can be cooled to almost absolute zero of temperature, or the flow of photons, the very constituents of light, can be tailored at will.
We apply this high level of control to applications ranging from the realisation of quantum simulators which provide us with a better understanding of quantum mechanics in certain biological processes, to the engineering of more accurate optical instruments. These can be microscopic probes but also bigger scientific instruments aiming to unlock some of the most tantalising scientific mysteries such as the existence of dark matter.
Our experimental techniques encompass laser physics, modern optics and optical detection, vacuum technology, electromagnetism, computer simulations and much more. We run our own table-top experiments and we take part in bigger collaborations, for instance within the Quantum Technology Hub or to develop the next generation of large instruments for fundamental physics. The breath of applications of our research leads us to collaborate with astrophysicists, electrical engineers, chemist and biochemists.
The quantisation of light into discrete quanta of energy, the photons, is responsible for the emergence of quantum noise caused by the random behaviour of these photons. This is because in a "normal" beam of light, photons propagate independently. It is possible to prepare a beam of light where the photons propagate in unison, in a correlated fashion, thereby reducing, or "squeezing", the quantum noise. We are interested in controlling the distribution of photons transversally with respect to the direction of propagation to shape the quantum properties of the beam profile, creating so-called "quantum images."
This is achieved by making laser light interact with a vapour of rubidium atoms. Nonlinear interaction introduces correlations between the light in different regions of space, a phenomenon called "quantum entanglement." For instance two regions can have an undefined yet equal number of photons!
We are studying the production of quantum entanglement and squeezing in space, and we are developing protocols and experiments to demonstrate their use to improve optical measurement and sensing techniques. This can be an imaging system or an instrument using the spatial fluctuations of laser, for instance an atomic magnetometer.
Light generated by lasers is at the core of many instruments and diagnostics. For instance it enables atomic force microscopy, which can image surfaces at the single atom level. It will also be used in the next generation of quantum magnetometers, which will help imaging the functioning brain and detecting dangerous substances inside sealed containers. Even the best of laser-based instruments are limited by the irregular flow of photons, the elementary constituents of light. This creates quantum fluctuations, also called shot noise . Our technology produces squeezed light, that is to say it regulates the flow of photons and makes these instruments more sensitive and accurate, delivering better and faster diagnostics and images.
Beam deflection measurement can be used to determine the tilt of an object. A prime example is the cantilever in an atomic force microscope. Our portable source of squeezed light goes around the limitation imposed by the shot noise and generates a better signal-to-noise ratio and enables the observation of motion, which may otherwise be invisible.
Quantum mechanics rules the behaviour of atoms and molecules. It usually becomes less relevant for bigger physical systems, and this is why we do not directly observe quantum behaviour in our daily life. It is therefore with surprise that researchers looking at energy transport in biological system, for instance during photosynthesis, observed that it seems to draw from properties that belong to both the quantum and the classical worlds.
Directly studying these energy transport networks in biological systems is challenging, and physicists are now seeking to use simpler systems that display the same behaviour. This way they can get a better understanding of how quantum mechanics can effectively interface with classical physics to provide efficient energy transport. This research seeks to use the internal state of single atoms to simulate these energy networks. It uses lasers to trap and cool down atoms so that they can be easily manipulated and observed with state-of-the-art laser spectroscopy. By looking at how the state of the atoms change when they are subject to external electromagnetic perturbations, one can answer some of the questions around efficient energy transport in quantum systems subject to noise. These answers have implications on how to build quantum systems that should not be sensitive to external perturbation, such as quantum computers. They also provide a glimpse into how evolution may have optimise the use of quantum effects in certain biological systems.
There are many theories that try to explain the nature of dark matter. Analyses of a range of observations, including the rotation velocities of galaxies, the dynamics of galaxy clusters, microlensing, and the large-scale structure of the universe led most of the scientific community to accept non-baryonic particles as the primary dark matter candidates.
The physical principle of axion-like-particle searches pursuits by our group is to explore a phase velocity difference between left- and right-handed circularly polarized light which propagates in the presence of axion-like-particle fields. We are building a quantum-enhanced interferometer to measure the phase difference induced by axions with masses from 10 -16 eV up to 10 -8 eV.
The sensitivity of the interferometer will be largely increased thanks to the use of quantum light, featuring reduced quantum fluctuations.
This is a collaborative project between the universities of Birmingham, Cardiff, Glasgow, Strathclyde and Warwick.
+44 (0)121
414 4573
v.boyer (at) bham.ac.uk
university page
CXF512
(at) student.bham.ac.uk
Quantum simulations
JXB1342 (at) student.bham.ac.uk
Super-resolved microscopy
NXM988
(at) student.bham.ac.uk
Quantum imaging
Quantum imaging, Squeezing for instrumentation
Quantum imaging
For all enquiries contact Vincent Boyer.
We are located on the Edgbaston campus of the University Birmingham. Travel to and navigate through the campus.
Our offices are on the second floor of Physics East, building R10 on the campus map.
Coming by rail? Take a train for Birmingham New Street and change there to catch a service in the direction of Bromsgrove or Redditch (usually platform 11 or 12). Get off at "University", turn left when exiting the station, and walk across the campus, past the clock tower, to the physics department.
Find us on Google maps.